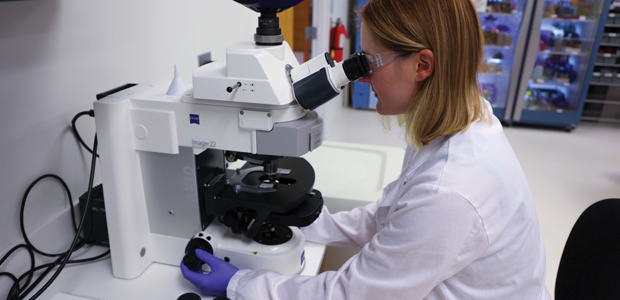
Oil Field Chemistry
Bio-Based Chemistries Pair Strong Performance With Green Characteristics
By Amir Mahmoudkhani and Jonathan Rogers
When Thomas Edison patented his incandescent light bulb in 1879, the primary market for oil—or then kerosene, which was used for lighting—nearly disappeared. But with the advent of the internal combustion engine, oil found a new market in transportation, which still dominates demand. The oil and gas industry’s history has been one of continuous challenges, matched by adaption and innovation. Technological advances constantly have shaped and reshaped every stage of the industry, from exploration right through to final usage.
Biology and chemistry are a pivotal part of every global industry–from agriculture, cooking and food production to personal health care products and consumer packaging. These industries excel in selecting and deploying highly effective microorganisms to produce chemicals that deliver results. What is not commonly known is that many of the ingredients from products used daily by consumers have made their way into the oil and gas industry, where they are shaping the future of oil field operations and management.
Enzymes
Some of the ingredients entering the oil field are enzymes, which are proteins produced by living cells that catalyze specific chemical reactions, increasing reaction frequency up to millions of times the norm. In humans, enzymes aid digestion, metabolize and eliminate waste from our bodies, and play a crucial role in muscle contraction. For millennia, they have been an essential part of cheese making, brewing and baking.
Improved methods for producing enzymes and the ability to dramatically change their properties through genetic engineering have opened the door to new applications in a wide range of industries. They have tremendous potential to reduce costs, produce less waste, and help decrease the need for energy, water and toxic chemicals.
Enzymes are studied for use in industrial-scale catalysis because they offer several advantages over traditional catalysts, from their ability to function in milder reaction conditions, e.g. to facilitate reactions at low temperatures, to their exceptional product selectivity and lower environmental and physiological toxicity. There are more than 3,000 known enzymes, but only 150-170 are in use commercially.
Enzymes are used in the food, agricultural, cosmetic and pharmaceutical industries in order to increase the reaction rates, yield and purity of the finished products. Breweries could not make beer without enzymes and the yeasts that produce them.
Industrial uses for enzymes are increasing with applications in the production of biofuels and biopolymers. Enzymes are transforming the nonfood industrial sectors to improve process efficiencies and decrease energy usage. For example, acrylamide, a building block for manufacturing a wide range of polymers and copolymers, including water treatment polymers, friction reducers and scale inhibitors, is made from acrylonitrile using nitrile hydratase. This enzyme efficiently converts acrylonitrile into acrylamide under mild conditions and offers significant improvements in yield efficiency and reduced energy needs compared with traditional chemical techniques.
Enzymes are also improving the production process for hydrogen peroxide, a simple molecule used as an oxidizer, bleaching agent and antiseptic. For more than 75 years, hydrogen peroxide has been synthesized through a single, complex chemical process that involves a succession of hydrogenation and oxidation reactions from a palladium catalyst. Certain enzymes have long been known to work with hydrogen peroxide in various biological systems.
Translating that knowledge into a biological-based method to create hydrogen peroxide proved difficult–until recently. A specialty chemicals manufacturer has developed and deployed the world’s first and only bio-based chemical peroxide. Solugen’s proprietary enzymes produce bio-based peroxides that are in use to treat, clean and oxidize several industries’ waste waters, including oil field produced water.
Enzyme-Based Breakers
Efficiently recovering hydraulic fracturing fluid normally means reducing the fluid’s viscosity after the frac using a breaker chemical. The breaker’s main purpose is to reduce the molecular weight of the completion fluid’s viscosifying agent, thereby minimizing viscosity and maximizing both the volume and rate of fluids returning from the formation. Misapplication or use of an ineffective breaker can cause significant damage in the proppant pack and reduced permeability.
Common frac fluid breakers include chemical oxidizers such as hydrogen peroxide and persulfates or organic acids. However, enzyme-based breakers such as hemicellulase, cellulose, amylase and pectinase have been widely used in water-based fracturing fluids for three decades.
Enzyme breakers have several advantages over traditional oxidizer chemicals. First, enzymes specifically break long-chain polymers without causing undesirable damage to the wellbore, formation or fracturing equipment. Second, because the enzymes are catalysts, they are not consumed in the breaking process and work well even in low concentrations. Third, enzymes are nontoxic compared with oxidant breakers.
As an example, the enzyme mannanase effectively can break linear and borate crosslinked guar under broad ranges of temperature and pH. This breaker belongs to the glucanase family and reduces gel viscosity by specifically targeting ß-1,4 glycosidic bonds between the mannose units in guar.
Enzyme-based breakers can work well in situations where chemical oxidizers struggle. For example, chemical oxidizers have trouble breaking or degrading the polyacrylamide-based friction reducers frequently used in slickwater completions. Asparaginase, an enzyme derived from a fungus used in East Asia for soybean fermentation, has proven to be far more effective.
Bio-Polymers
Bio-based polymers are materials produced from renewable resources. The terms bio-based polymers and biodegradable polymers are used extensively in literature, but there is a key difference between them. Biodegradable polymers are materials whose physical and chemical properties deteriorate and eventually degrade completely when exposed to microorganisms, aerobic and anerobic processes or hydrolysis. Bio-based polymers can be degradable (e.g. polylactic acid) or nondegradable (e.g. biopolythioesters). Similarly, while many bio-based polymers are biodegradable (e.g. starch and polyhydroxyalkanoates), not all biodegradable polymers are bio-based (e.g. polycaprolactone).
Bio-based polymers are attracting increased attention motivated by environmental concerns and the realization that global petroleum resources are finite and more renewable, sustainable materials must be identified. Bio-based polymers not only replace existing polymers in several applications, but also provide new combinations of properties that unlock new applications.
Bio-based polymers typically are produced through three methods: 1) extraction and separation from agricultural resources, 2) fermentation processes and 3) conventional synthesis using building blocks from renewable resources, including lignocellulosic biomass, fatty acids and organic waste. Some bio-based polymers, such as proteins, nucleic acids and polysaccharides, occur naturally.
One of the more commercially intriguing bio-based polymers is polylactic acid (PLA). Although it was discovered in 1845, PLA was not commercialized until the early 1990s. Its unique and appealing characteristics include good transparency, a glossy appearance, high rigidity and the ability to tolerate various processing conditions.
PLA is widely used in food packaging, including food trays, water bottles, candy wrappers and tea bags. More recently, it has been employed as a membrane material in the automotive and chemical industries. In its oil field incarnations, the polymer appears in fluid loss control and fluid diverting agents, frac sand and proppant carrier agents, filter cake removers and degradable objects.
Like PLA, polyhyroxyalkanoates (PHAs), a family of polyesters produced by bacterial fermentation, have migrated to the oil field. PHAs occur naturally in a variety of organisms. The most efficient producers can be isolated and commercially-fermented to maximize the PHA’s quality and yield.
Polyhyroxybutyrate, the simplest PHA, was discovered in 1926 by Maurice Lemoigne as a constituent of the bacterioum bacillus megaterium. Additional PHA monomers have since been identified, and this diversity allows the production of multiple bio-based polymers with a wide range of properties tailored for specific applications.
PHA possesses similar thermal and mechanical properties to polystyrene and polypropylene. PHAs are therefore suitable for a wide range of food and medical packaging applications, as well as disposable items such as hot cup lids, forks, spoons, knives and cosmetics. PHA and its copolymers are widely used as biomedical implant materials and drug delivery systems. In the oil industry, PHAs are used for filter cake removal, as internal breakers to decrease the viscosity of completion fluids, and to encapsulate chemical additives for controlled chemical release applications.
Biosolvents
Solvents are a vital ingredient of many chemical processes and products. They are used in a variety of industrial applications, including agrochemicals, paints and coatings, household and industrial cleaners and pharmaceuticals. Nowadays, consumers can choose solvents not only based on their physical properties, but also on their environmental impacts. Many consumers prefer eco-friendly solvents or green substitutes for conventional products. In this new era, biosolvents are becoming more widely used because they pair eco-friendly characteristics with lower costs.
For a solvent to be considered green, it must meet several criteria. It should be bio-based, free of volatile organic compounds, nontoxic, biodegradable and recyclable. Green solvents can include a variety of products (Figure 1), especially bio-based ethanol, D-limonene, dipentene, and methyl soyate.
While methyl soyate (soy fatty acid methyl esters) is gaining market share rapidly, many manufacturers currently prefer bio-based ethanol because its widespread commercialization makes it a more affordable and convenient purchase. It helps that bio-based ethanol is suitable for a multitude of applications. Ethyl lactate is another green solvent that is growing in popularity, primarily as a replacement for petroleum solvents.
Recently, the Circa Group, a company that offers solutions for converting waste biomass into advanced biochemical materials, has introduced a bio-based dipolar aprotic solvent, Cyrene, that offers an alternative to petroleum-derived dimethylformamide and N-Methyl-2-pyrrolidone products. These products are used to produce pharmaceuticals, pigments, paint strippers and adhesives.
One of the more exciting use cases for green solvents is in dry cleaning. Traditional dry cleaning solvents produce harmful waste products including carbon, dyes, grease and various filter materials. But thanks to Green Biologics, dry cleaners now have access to a bio-based solvent (methylene glycol dibutyl ether) made from corn. Called SOLVONK4, it cleans better than traditional solvents, does not harm the environment and minimizes health-related concerns. Moreover, it is the first and only bio-based solvent to be used for dry cleaning.
Various biosolvents, including D-limonene, dipentene, lactic acid esters and fatty acid esters, have been used in the oil field to improve efficiency in oil-based drilling muds and remediation and stimulation fluid systems. Such fluid systems are helping remove pipeline deposits, remediate paraffin and asphaltene deposits and clean tanks.
Bio-based solvents have been employed in the formulation and delivery of production chemicals as well as products for drilling and completion applications. For example, they often are paired with surfactants to obtain microemulsions for flowback aids and to enhance oil recovery from conventional and unconventional reservoirs.
Surfactants
Surfactants, molecules that adhere to interfaces (e.g., water-oil, liquid-gas, and solid-liquid or solid-gas) and lower their surface energy, have numerous applications in our everyday lives, including foods, medicines, toiletries, cleaners, automotive fluids, paints and coatings, and processing aids. Their surface activity is enabled by their molecular structure, consisting of separated hydrophilic and lipophilic domains.
The European Commission of Standardization (CEN) derived a classification of bio-based surfactants based on their bio-based content (i.e., percentage of carbon atoms derived from renewable resources): wholly bio-based (>95%), majority bio-based (50%–94%), minority bio-based (5%–49%), and nonbio-based (<5%). Bio-based surfactants play a role in most market sectors associated with surfactants: laundry detergents, foods, cosmetics, personal care products, pharmaceuticals, paints and coatings, oil and gas and environmental remediation, to name a few.
Significant public, scientific and political focus has been given to the environmental impacts caused by synthetic, hydrocarbon-based surfactants in the oil industry, such as oil dispersants used to clean up offshore oil spills. These concerns center on the surfactants’ toxicity and long life in the environment. Advances in biotechnology and the emergence of more stringent guidelines on chemical use have led to biosurfactants becoming a viable alternative to chemical surfactants.
Biosurfactant research, development and commercialization has accelerated dramatically. However, there is no clear definition of the term “biosurfactant” in literature. Often, it is used synonymously when referring to amphiphilic secondary metabolites secreted by microorganisms or for surfactants that are degradable naturally or produced from renewable resources.
The most common biosurfactants in the broadest sense are alkyl polyglucosides (APGs) that are condensed chemically by dehydration of sugar and fatty alcohols. Additionally, there are other natural surfactants, such as saponins from vegetable sources (e.g., extracts of the soapberry, sapindus saponaria) or lecithins from animal sources (e.g., egg yolk lecithin) that are often utilized in the food industry.
Microbial Surfactants
Microbial surfactants are biosurfactants produced from microbial cultures. These often are secreted by microorganisms into the culture medium when grown on hydrophobic carbon sources. The primary reason for the interest in microbial biosurfactants is the idea of replacing or complementing petroleum-based surfactants in industrial and consumer applications. This is mainly from their claimed low ecotoxicity combined with their complete biological degradability and production from renewable products.
Although biosurfactants have been known for several years to be promising for use in bioremediation processes, industrial-scale production previously had been limited by high raw-material and processing costs and low manufacturing output. Using advanced fermentation techniques developed by companies such as Locus Bio-Energy Solutions, the production constraints have been addressed. In fact, viable quantities of biosurfactants now can be produced at costs comparable with or even more attractive than traditional synthetic surfactants.
Several studies show that biosurfactants can replace virtually any synthetic surfactant and, moreover, introduce unique, advantageous physicochemical properties. For now, the primary oil field applications for biosurfactants are enhancing oil recovery, remediating hydrocarbons and dispersing wax deposits, applications that benefit from the biosurfactants’ biodegradability, excellent surface wettability and extremely low critical micelle concentration.
In enhanced oil recovery applications, advanced biosurfactants have delivered production increases between 50% and 400%. The biosurfactants have proven consistent enough that they have become the first technology to be named New Technology of the Year by Oil & Gas Awards in both the Northeast and Texas regions.
Microorganisms produce amphiphilic metabolites displaying considerable structural diversity. Most biosurfactants are low-molecular-weight microbial amphiphiles that consist of a hydrophilic head group and a hydrophobic tail and can include a diversity of subunits such as sugars, fatty acids, amino acids and carboxylic acid groups. Glycolipids comprise the greatest share of commercially-available microbial surfactants. Besides glycolipids, lipopeptides are another class of biosurfactants of high commercial and scientific interest.
Sophorolipids
Sophorolipids (SLs) are glycolipid biosurfactants produced by certain yeast species from renewable resources. Typically, natural SLs are composed of a complex mixture of lactonic and acidic varieties with variations in the acetylation patterns at position 6’ and/or 6” of the constituting glucose moieties, in the identity of the hydrophobic portion of the molecule, and in its linkage to the hydrophilic (sophorose) sugar (Figure 2).
SLs were first discovered in 1961 as metabolites from the yeast torulopsis magnoliae. SLs have numerous potential applications. Research shows they can be effective additives in cleaning supplies and in agriculture, food, pharmaceuticals, cosmetics and enhanced oil recovery. The most touted uses are in surfactant application areas. While the main surfactant applications of interest are as cleaning agents and emulsifiers, SLs could also be solubilization agents, wetting agents or antifoaming agents.
Biosurfactants have been applied in many oil industry domains, including petroleum production and incorporation into oil field chemical formulations, which likely is the largest possible market. Other applications related to the industry include oil spill bioremediation/dispersion, both inland and at sea, bioremediation of nonaqueous phase liquids, removal/mobilization of oil sludge from storage tanks and EOR.
A recent study demonstrates how well sophorolipids reduce surface and interfacial tension with a high emulsification index against various hydrocarbons, including light and heavy crude oils, while offering high stability under extreme conditions of salinity, pH and temperature. Core-flooding studies reveal that SLs can recover 27.27% of residual oil trapped between the pores of Berea sandstone cores, highlighting SLs’ potential to yield great results in enhanced oil recovery applications.
The evolution of chemistry in top global industries has found its way into useful applications for all phases of upstream oil and gas, from improving drilling and completion performance to boosting and maintaining production. Advances in bio-genomics, biochemistry and fermentation processes are allowing us to, as the 1944 Bing Crosby song advised, “accentuate the positive and eliminate the negative” and apply the power of microorganisms to oil and gas applications.
A key advantage of microbial fermentation is that the components required to grow the host strain and synthesize the product can be derived from inexpensive sources of carbon, nitrogen, trace elements and energy. Provided the cells grow rapidly and allow for easy extraction of the target chemical, microbial fermentation can reduce costs significantly for producing pure, high-quality chemicals. Furthermore, bio-producing these fine chemicals typically requires lower temperatures than chemical syntheses and involves processes that are not hazardous to the environment.
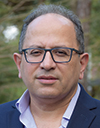
AMIR MAHMOUDKHANI is director of technology for Locus Bio-Energy Solutions, and is responsible for product and application development at the company’s innovation center in Houston. With strong knowledge of oil field chemistry, 18 years of experience in various technical roles, and service as an adjunct professor at the University of Alberta from 2011-2015, Mahmoudkhani has 126 publications and is an inventor and co-inventor of 38 patent applications in oil and gas. He holds a doctorate in materials chemistry from Sweden’s Göteborg University.
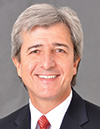
JONATHAN ROGERS is Locus Bio-Energy Solutions’ chief executive officer. He has 30 years of oil and gas experience, previously working as the global head of Clariant’s oil field operations, global technology director for BP, executive vice president at Champion Technologies and managing director at Sasol Oilfield Chemicals. Rogers is a fellow of the Royal Society of Chemistry and a holder of four patents.
For other great articles about exploration, drilling, completions and production, subscribe to The American Oil & Gas Reporter and bookmark www.aogr.com.